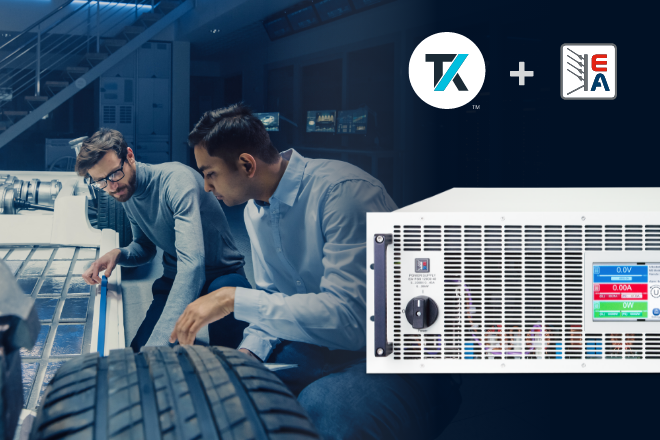
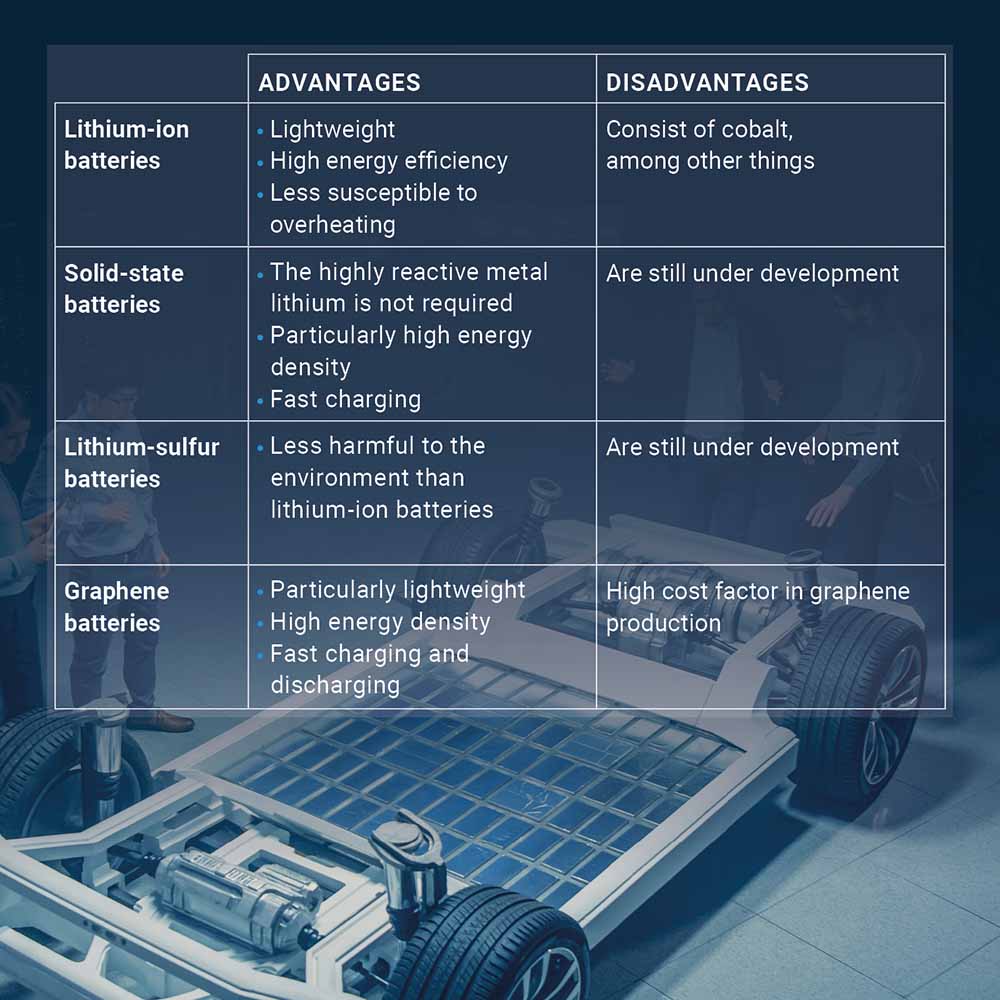
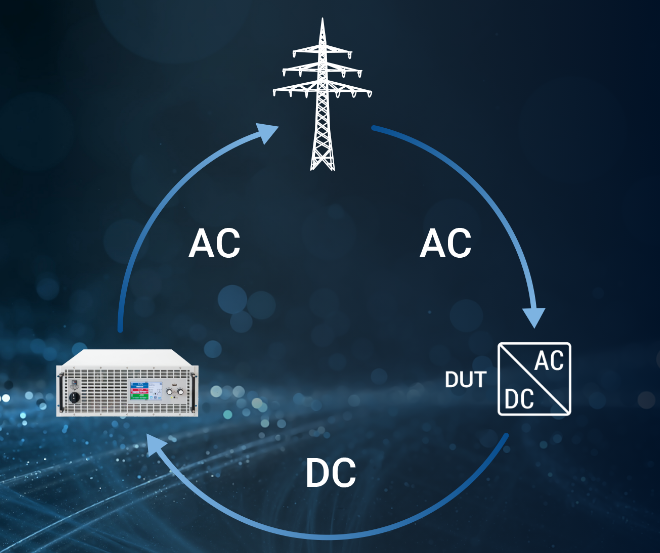
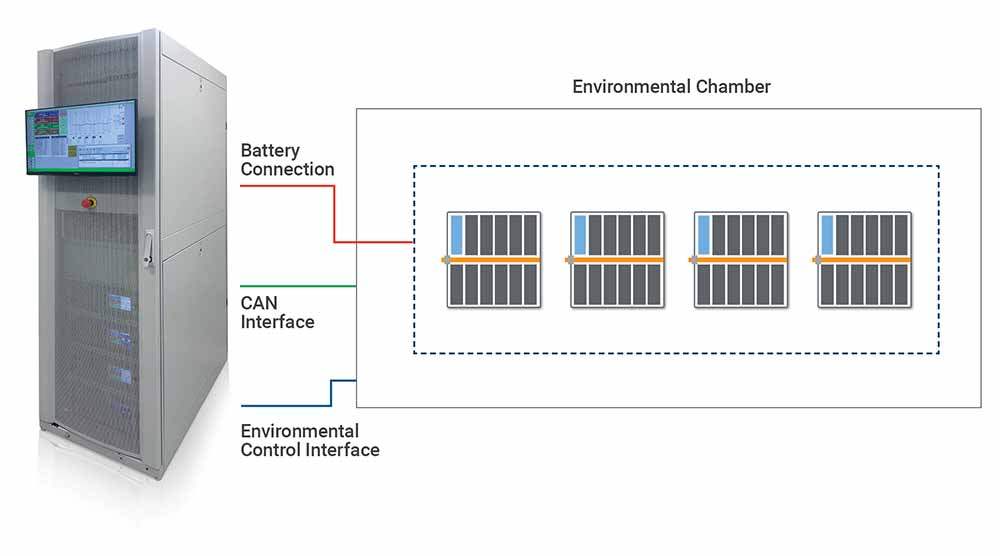
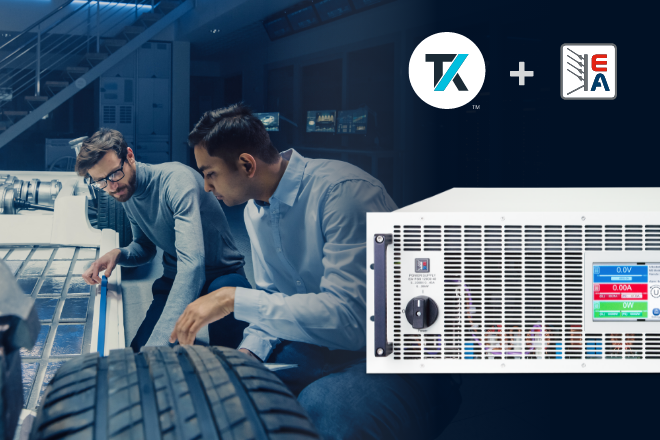
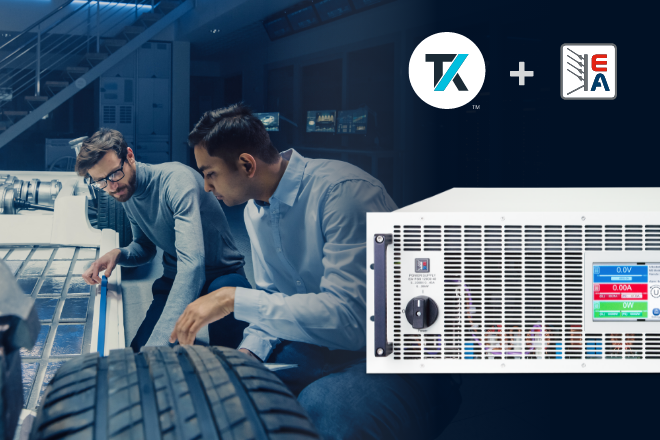
Electric vehicle battery manufacturers and EV manufacturers are working to overcome the two biggest challenges of electric mobility: range and charging time. Within this framework, testing systems must be flexible to adapt to higher voltages and higher-capacity batteries. The following sections provide an overview of current and future battery technologies for electric vehicles. It then examines how battery test systems can keep pace with the development of new battery packs.
EV battery technologies
Lithium-ion batteries are comparatively lightweight, exhibit high energy efficiency, and are less likely to overheat at high temperatures. Compared to other battery types, Li-ion takes longer to discharge, thereby offering travel ranges up to 500+ miles on a full charge. In North America and Europe, EV Li-ion batteries employ either nickel, manganese, and cobalt (NMC) or nickel, manganese, cobalt, and aluminum (NMCA). Widely used in China, lithium-iron-phosphate (LFP) exhibits lower energy densities, which require larger battery sizes to provide energy levels comparable to NMC-based batteries.
Under development, solid-state batteries show great promise for use in future EVs. Solid- state batteries can avoid the use of the highly reactive metal lithium. Electrolytes are solid materials such polymers, ceramics or glass. Anodes can be graphite or silicon, and cathodes can be nickel-based materials. Solid-state batteries require less space and offer higher energy densities than Li-ion batteries. As a result, solid-state batteries could double the range of electric vehicles. The solid-state electrolyte permits ions to move more quickly between the battery anode and cathode, enabling faster charging.
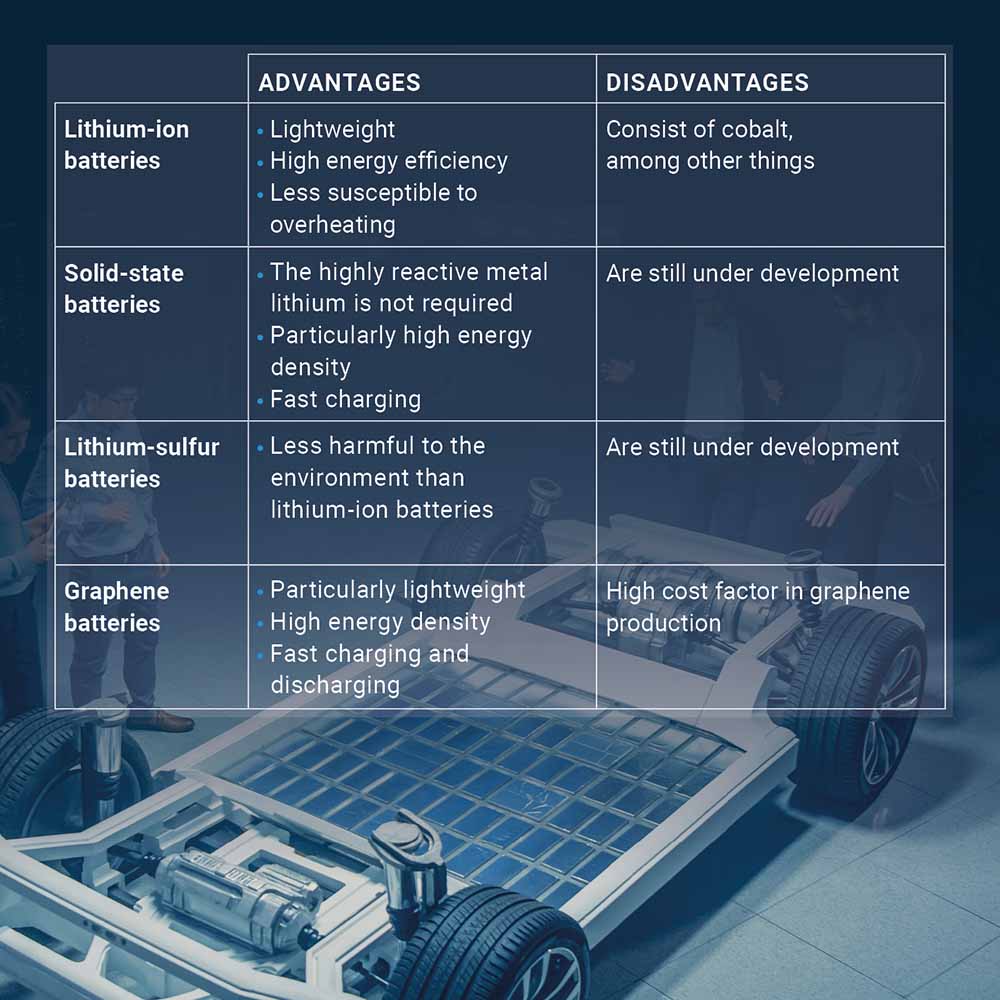
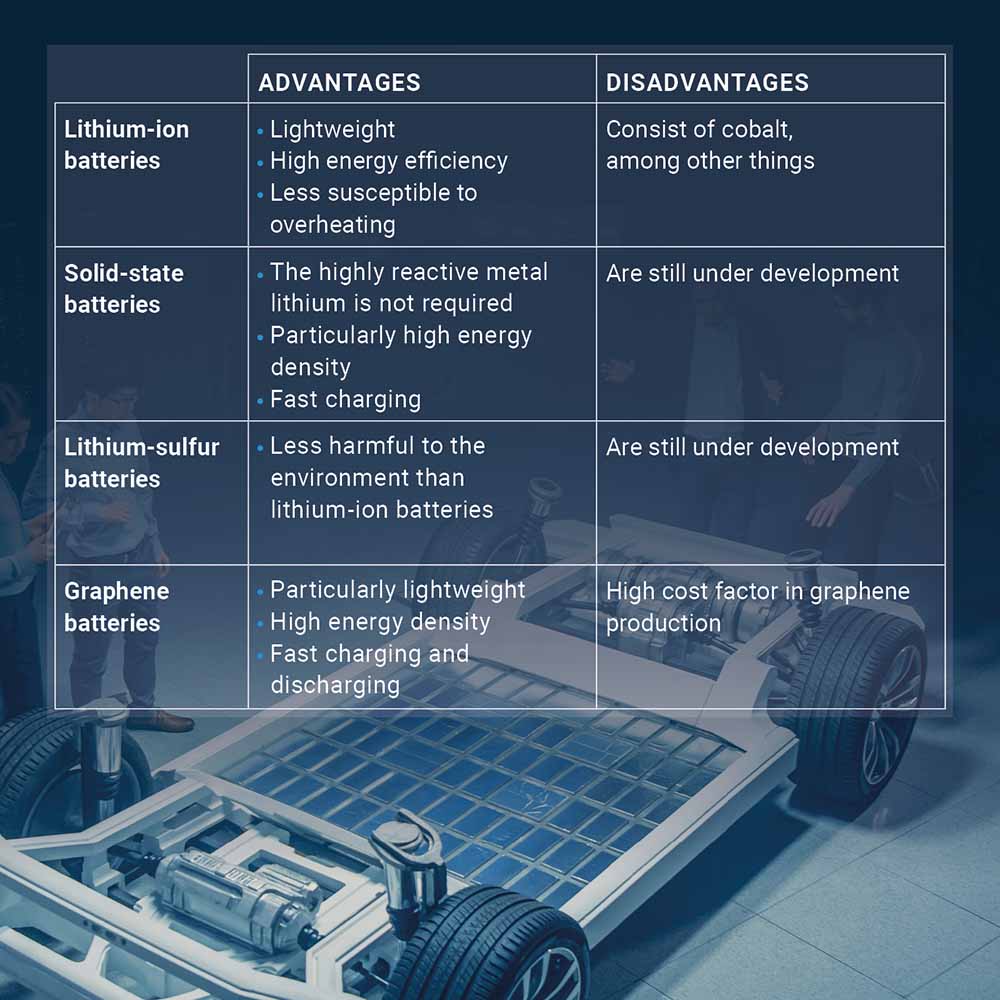
Another future alternative to Li-ion, lithium-sulfur, is similar to solid state. Solid-state batteries offer a longer range than Li-ion batteries and are less costly to produce. The belief in Europe is that lithium-sulfur batteries present less of an impact on the environment compared to Li-ion batteries made of cobalt.Â
Compared to Li-ion batteries, graphene batteries are lighter, physically stronger, and dissipate heat better during the charge/discharge cycle, limiting the risk of overheating and fires. Additionally, graphene batteries can store larger amounts of energy in the same size package and recharge faster. Significant challenges to mainstream adoption include scalability of graphene production, cost-effectiveness, and integration into existing battery manufacturing processes.
Said to be inexpensive and powerful, zinc-air batteries deliver power when oxygen oxidizes zinc. However, oxidation degrades battery performance, a barrier to wide use of zinc-air batteries.
Increasing battery voltage and total kWh capacity
EV battery packs are increasing in voltage and capacity to address the travel distance challenge. Some vehicles on the market have 800 V battery packs. Large vehicles such as trucks, trains, and airplanes will soon employ even higher battery voltages. Higher voltages reduce the current requirements, which reduces the size of the current-carrying wires. Smaller wiring assemblies reduce vehicle weight, contributing to greater vehicle efficiency and longer drive distance.Â
An 800 V battery requires over 200 Li-ion 4.2 V cells, yielding a nominal voltage of 830V. The voltage on the battery can rise an additional 20 to 30 V during regenerative breaking, resulting in a battery voltage that can reach 860 V. Having a switching power supply, for example, with a flyback converter topology, to charge the test system requires a circuit that is 150 V to 200 V higher than the battery voltage. Next, add in 20% headroom, and the circuit can have a maximum voltage of around 1.3 kV. At that voltage, a test system will need a source voltage capacity of 1500 to 2000 V to test and charge 800 V and higher battery packs. Â
In addition to higher voltage EV batteries, battery capacity is increasing as well. At least one manufacturer is using a 100 kWh battery pack to extend drive distance. To reduce recharge time, companies are developing 250 kW charging systems that can add up to 180 miles of drive distance in 15 to 20 minutes.Â
The testing challenge
Battery test systems need to evolve to test the higher voltage and higher capacity EV battery packs. As indicated, test systems will need voltages to exceed 1000 V and power over 250 kW capacity to test a battery’s maximum voltage and its ability to accept a high current charge rate. A high-voltage and high-power system will enable battery cycling to ensure the battery pack meets its voltage-time discharge curve specification and to estimate cycle life under fast charging conditions.Â
Other tests include:
- Pulse tests that require the battery to deliver or absorb high current in short intervals for the assessment of battery thermal design and state of charge (SOC)
- Drive cycle simulation to conform to standard drive simulation tests such as Federal Test Procedure (FTP-75)
- DC internal resistance to assess a battery’s state-of-health (SOH)
- Insulation resistance to identify defects that can reduce battery efficiency and cause safety issues.Â
Some test systems need to be capable of vibration and shock testing, which is essential for the reliability testing of off-road vehicles. However, this testing is a quality assurance test rather than a production test performed on all battery packs.Â
Batteries can be operated in cycles with bidirectional power supplies. Devices such as those manufactured by EA Elektro-Automatik, have power levels as high as 30 kW and 60 kW. With these supplies, one 42U high test rack can have a 300 kW capacity while consuming only 6.5 sq ft of manufacturing floor space. With EA supplies having a unique true autoranging output (or input) that allows delivery (or absorption of full power down to 1/3 of the supply’s rated voltage, one 300 kW rack can support 2.4 kA.Â
Testing large, high-capacity battery packs necessitates that a battery test system has protection features to prevent damage to battery packs and the test system. Systems need to monitor battery temperature, provide safety checks to prevent reverse polarity connections, and ensure the battery is sufficiently pre-charged to a safe level before it can accept a large charge current.Â
At such high-power levels, the bidirectional power supplies (or electronic loads) can save substantial utility costs if they are regenerative and efficiently return absorbed power to the AC grid. For example, EA bidirectional power supplies and loads can return absorbed energy with exceptional efficiency—up to 96.5%.
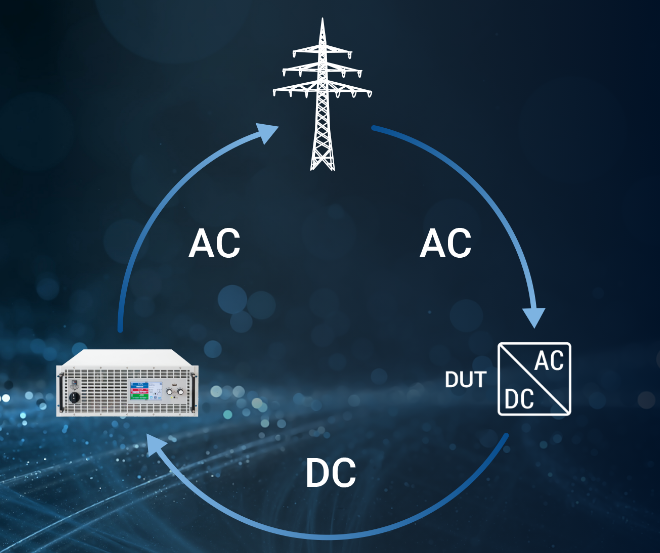
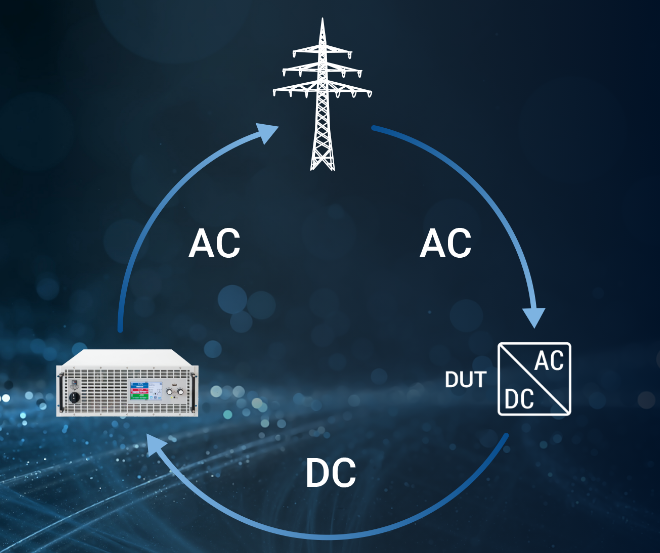
Performing the pulse tests and drive cycle simulations requires a test system with fast slew rates and high data acquisition rates for data capture. The systems also need waveform generation capability for the drive cycle simulations.Â
With growing production volumes, increasing throughput is critical. While the battery pack dictates the speed of testing, more test capacity can generate increased throughput. A system that can increase power capacity into the MW range can increase the number of batteries tested simultaneously to increase throughput. Further details can be found in our white paper, which you can download free of charge.
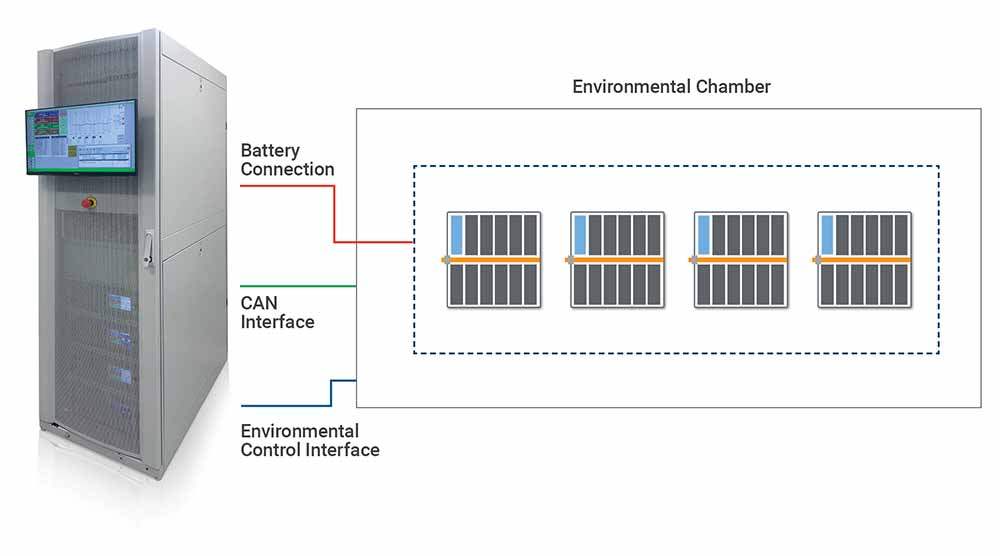
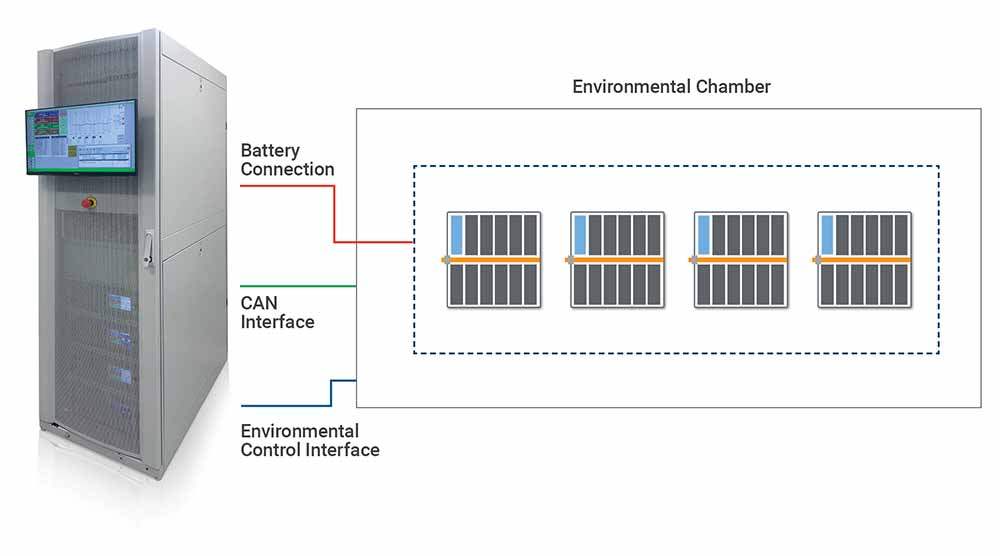
Requirements for the battery test system manufacturers
New EV batteries will be coming out of the lab and onto the market soon. They will have higher voltages, higher capacity, and the ability to recharge quickly. EV battery test systems will need to have the voltage levels, amounts of power, safety features, and throughput to test the new batteries for a growing EV market. These test systems will need a return on investment from utility cost savings and manufacturing overhead savings. EA is one battery test system manufacturer that is addressing these challenges.Â

Source link
#Futureproof #battery #cycler #test #system #Whitepaper